User:Jhurley/sandbox
Downscaled High Resolution Datasets for Climate Change Projections
Global climate models (GCMs) have generated projections of temperature, precipitation and other important climate change parameters with spatial resolutions of 100 to 300 km. However, higher spatial resolution information is required to assess threats to individual installations or regions. A variety of “downscaling” approaches have been used to produce high spatial resolution output (datasets) from the global climate models at scales that are useful for evaluating potential threats to critical infrastructure at regional and local scales. These datasets enable development of information about projections produced from various climate models, about downscaling to achieve desired locational specificity, and about selecting the appropriate dataset(s) to use for performing specific assessments. This article describes how these datasets can be accessed and used to evaluate potential climate change impacts.
Related Article(s):
Contributor(s): Dr. Rao Kotamarthi
Key Resource(s):
- Use of Climate Information for Decision-Making and Impacts Research: State of our Understanding[1]
- Applying Climate Change Information to Hydrologic and Coastal Design of Transportation Infrastructure, Design Practices[2]
- Statistical Downscaling and Bias Correction for Climate Research[3]
- Downscaling Techniques for High-Resolution Climate Projections: From Global Change to Local Impacts[4]
Downscaling of Global Climate Models
Some communities and businesses have begun to improve their resilience to climate change by building adaptation plans based on national scale climate datatsets (National Adaptation Plans), regional datasets (New York State Flood Risk Management Guidance[5]), and datasets generated at local spatial resolutions. Resilience to the changing climate has also been identified by the US Department of Defense (DoD) as a necessary part of the installation planning and basing process (DoD Report on Effects of a Changing Climate[6]). More than 79 installations were identified as facing potential threats from climate change. The threats faced due to changing climate include recurrent flooding, droughts, desertification, wildfires and thawing permafrost.
Assessing the threats climate change poses at regional and local scales requires data with higher spatial resolution than is currently available from global climate models. Global-scale climate models typically have spatial resolutions of 100 to 300 km, and output from these models needs to be spatially and/or temporally disaggregated in order to be useful in performing assessments at smaller scales. The process of producing higher spatial-temporal resolution climate model output from coarser global climate model outputs is referred to as “downscaling” and results in climate change projections (datasets) at scales that are useful for evaluating potential threats to regional and local communities and businesses. These datasets provide information on temperature, precipitation and a variety of other climate variables for current and future climate conditions under various greenhouse gas (GHG) emission scenarios. There are a variety of web-based tools available for accessing these datasets to evaluate potential climate change impacts at regional and local scales.
Methods for Downscaling
Dynamical Downscaling | Statistical Downscaling |
---|---|
Deterministic climate change simulations that output many climate variables with sub-daily information |
Primarily limited to daily temperature and precipitation |
Computationally expensive; hence, limited number of simulations – both GHG emission scenarios and global climate models downscaled |
Computationally efficient; hence, downscaled data typically available for many different global climate models and GHG emission scenarios |
May require additional bias correction | Method incorporates bias correction |
Observational data at the downscaled location are not necessary to obtain the downscaled output at the location |
Best suited for locations with 30 years or more of observational data |
Does not assume stationarity or in other words the model simulates the future regardless of what has happened in the past |
Stationarity assumption - assumes that the statistical relationship between global climate model and observations will remain constant in the future |
There are two main approaches to downscaling. One method, commonly referred to as “statistical downscaling”, uses the empirical-statistical relationships between large-scale weather phenomena and historical local weather data. In this method, these statistical relationships are applied to output generated by global climate models. A second method uses physics-based numerical models (regional-scale climate models or RCMs) of weather and climate that operate over a limited region of the earth (e.g., North America) and at spatial resolutions that are typically 3 to 10 times finer than the global-scale climate models. This method is known as “dynamical downscaling”. These regional-scale climate models are similar to the global models with respect to their reliance on the principles of physics, but because they operate over only part of the earth, they require information about what is coming in from the rest of the earth as well as what is going out of the limited region of the model. This is generally obtained from a global model. The primary differences between statistical and dynamical downscaling methods are summarized in Table 1.
It is important to realize that there is no “best” downscaling method or dataset, and that the best method/dataset for a given problem depends on that problem’s specific needs. Several data products based on downscaling higher level spatial data are available (USGS, MACA, NARCCAP, CORDEX-NA). The appropriate method and dataset to use depends on the intended application. The method selected should be able to credibly resolve spatial and temporal scales relevant for the application. For example, to develop a risk analysis of frequent flooding, the data product chosen should include precipitation at greater than a diurnal frequency and over multi-decadal timescales. This kind of product is most likely to be available using the dynamical downscaling method. SERDP reviewed the various advantages and disadvantages of using each type of downscaling method and downscaling dataset, and developed a recommended process that is publicly available[1]. In general, the following recommendations should be considered in order to pick the right downscaled dataset for a given analysis:
- When a problem depends on using a large number of climate models and emission scenarios to perform preliminary assessments and to understand the uncertainty range of projections, then using a statistical downscaled dataset is recommended.
- When the assessment needs a more extensive parameter list or is analyzing a region with few long-term observational data, dynamically downscaled climate change projections are recommended.
Uncertainty in Projections
Aquifer Material | Total Porosity (dimensionless) |
Effective Porosity (dimensionless) |
Hydraulic Conductivity (meters/second) |
---|---|---|---|
Unconsolidated | |||
Gravel | 0.25 - 0.44 | 0.13 - 0.44 | 3×10-4 - 3×10-2 |
Coarse Sand | 0.31 - 0.46 | 0.18 - 0.43 | 9×10-7 - 6×10-3 |
Medium Sand | — | 0.16 - 0.46 | 9×10-7 - 5×10-4 |
Fine Sand | 0.25 - 0.53 | 0.01 - 0.46 | 2×10-7 - 2×10-4 |
Silt, Loess | 0.35 - 0.50 | 0.01 - 0.39 | 1×10-9 - 2×10-5 |
Clay | 0.40 - 0.70 | 0.01 - 0.18 | 1×10-11 - 4.7×10-9 |
Sedimentary and Crystalline Rocks | |||
Karst and Reef Limestone | 0.05 - 0.50 | — | 1×10-6 - 2×10-2 |
Limestone, Dolomite | 0.00 - 0.20 | 0.01 - 0.24 | 1×10-9 - 6×10-6 |
Sandstone | 0.05 - 0.30 | 0.10 - 0.30 | 3×10-10 - 6×10-6 |
Siltstone | — | 0.21 - 0.41 | 1×10-11 - 1.4×10-8 |
Basalt | 0.05 - 0.50 | — | 2×10-11 - 2×10-2 |
Fractured Crystalline Rock | 0.00 - 0.10 | — | 8×10-9 - 3×10-4 |
Weathered Granite | 0.34 - 0.57 | — | 3.3×10-6 - 5.2×10-5 |
Unfractured Crystalline Rock | 0.00 - 0.05 | — | 3×10-14 - 2×10-10 |
A primary cause of uncertainty in climate change projections, especially beyond 30 years into the future, is the uncertainty in the greenhouse gas (GHG) emission scenarios used to make climate model projections. The best method of accounting for this type of uncertainty is to apply a climate change model to multiple GHG emission scenarios (see also: Wikipedia: Representative Concentration Pathway).
The uncertainties in climate projections over shorter timescales, less than 30 years out, are dominated by something known as “internal variability” in the models. Different approaches are used to address the uncertainty from internal variability[4]. A third type of uncertainty in climate modeling, known as scientific uncertainty, comes from our inability to numerically solve every aspect of the complex earth system. We expect this scientific uncertainty to decrease as we understand more of the earth system and improve its representation in our numerical models. As discussed in Climate Change Primer, numerical experiments based on global climate models are designed to address these uncertainties in various ways. Downscaling methods evaluate this uncertainty by using several independent regional climate models to generate future projections, with the expectation that each of these models will capture some aspects of the physics better than the others, and that by using several different models, we can estimate the range of this uncertainty. Thus, the commonly accepted methods for accounting for uncertainty in climate model projections are either using projections from one model for several emission scenarios, or applying multiple models to project a single scenario.
A comparison of the currently available methods and their characteristics is provided in Table 2 (adapted from Kotamarthi et al., 2016[1]). The table lists the various methodologies and models used for producing downscaled data, and the climate variables that these methods produce. These datasets are mostly available for download from the data servers and websites listed in the table and in a few cases by contacting the respective source organizations.
The most popular and widely used format for atmospheric and climate science is known as NetCDF, which stands for Network Common Data Form. NetCDF is a self-describing data format that saves data in a binary format. The format is self-describing in that a metadata listing is part of every file that describes all the data attributes, such as dimensions, units and data size and in principal should not need additional information to extract the required data for analysis with the right software. However, specially built software for reading and extracting data from these binary files is necessary for making visualizations and further analysis. Software packages for reading and writing NetCDF datasets and for generating visualizations from these datasets are widely available and obtained free of cost (NetCDF-tools). Popular geospatial analysis tools such as ARC-GIS, statistical packages such as ‘R’ and programming languages such as Fortran, C++, and Python have built in libraries that can be used to directly read NetCDF files for visualization and analysis.
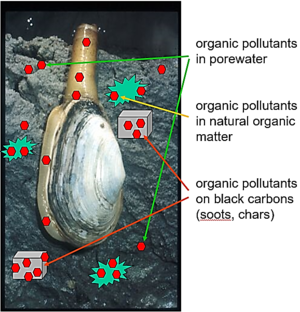
Environmental media such as sediments typically contain many different materials or phases, including liquid solutions (e.g. water, nonaqueous phase liquids like spilled oils) and diverse solids (e.g., quartz, aluminosilicate clays, and combustion-derived soots). Further, the chemical concentration in the porewater medium includes both molecules that are "truly dissolved" in the water and others that are associated with colloids in the porewater[7][8][9]. As a result, contaminant chemicals distribute among these diverse media (Figure 1) according to their affinity for each and the amount of each phase in the system[10][11][12][13][14]. As such, the chemical concentration in any one medium (e.g., truly dissolved in porewater) in a multi-material system like sediment is very hard to know from measures of the total sediment concentration, which unfortunately is the information typically found by analyzing for chemicals in sediment samples.
If an animal moves into this system, it will also accumulate the chemical in its tissues from the loads in all the other materials (Figure 1). This can lead to exposures of the chemical to other organisms, including humans, who may eat such animals. Predicting the quantity of contaminant in the animal requires knowledge of the relative affinities of the chemical for the animal versus the sediment materials. For example, if one knew the chemical's truly dissolved concentration in the porewater and could reasonably assume the chemical of interest in the animal has mostly accumulated in its lipids (as is often the case for very hydrophobic compounds), then one could estimate the chemical concentration in the animal (Canimal, typically in units of μg/kg animal wet weight) using a lipid-water partition coefficient, Klipid-water, typically in units of (μg/kg lipid)/(μg/L water), and the porewater concentration of the chemical (Cporewater, in μg/L) with Equation 1.
Equation 1. | Canimal = flipid x Klipid-water x Cporewater | |
where: | ||
flipid | is the fraction lipids contribute to the total wet weight of the animal (kg lipid/kg animal wet weight), and | |
Cporewater | is the freely dissolved contaminant concentration in the porewater surrounding the animal. |
While there is a great deal of information on the values of Klipid-water for many chemicals[15], it is often very inaccurate to estimate truly dissolved porewater concentrations from total sediment concentrations using assumptions about the affinity of those chemicals for the solids in the system[10]. Further, it is difficult to isolate porewater without colloids and/or measure the very low truly dissolved concentrations of hydrophobic contaminants of concern like polycyclic aromatic hydrocarbons (PAHs), polychlorinated biphenyls (PCBs), nonionic pesticides like dichlorodiphenyltrichloroethane (DDT), and polychlorinated dibenzo-p-dioxins (PCDDs)/ dibenzofurans (PCDFs)[16].
Passive Samplers
One approach to address this problem for contaminated sediments is to insert into the sediment billets of organic polymers like low density polyethylene (LDPE), polydimethylsiloxane (PDMS), or polyoxymethylene (POM) that can absorb such hydrophobic chemicals from their surroundings[17][18][19][20][21][22][23]. In this approach, the polymer is inserted in the sediment bed where it absorbs some of the contaminant load via the contaminant's diffusion into the polymer from the surroundings. When the polymer achieves sorptive equilibration with the sediments, the chemical concentration in the polymer, Cpolymer (μg/kg polymer), can be used to find the corresponding concentration in the porewater, Cporewater (μg/L), using a polymer-water partition coefficient, Kpolymer-water ((μg/kg polymer)/(μg/L water)), that has previously been found in laboratory testing[24][25], as shown in Equation 2.
Equation 2. | Cporewater = Cpolymer / Kpolymer-water |
Such “passive uptake” by the polymer also reflects the availability of the chemicals for transport to adjacent systems (e.g., overlying surface waters) and for uptake into organisms (e.g., bioaccumulation). Thus, one can use the porewater concentrations to estimate the biotic accumulation of the chemicals, too. For example, for the concentration in the animal equilibrated with the sediment, Canimal (μg/kg animal), would be found by combining Equations 1 and 2 to get Equation 3.
Equation 3. | Canimal = flipid x Klipid-water x Cpolymer / Kpolymer-water |
KPE-sed = CPE / Csediment.
Performance Reference Compounds (PRCs)
Perhaps unsurprisingly, pollutants with low water solubility like PAHs, PCBs, etc. do not diffuse quickly through sediment beds. As a result, their accumulation in polymeric materials in sediments can take a long time to achieve equilibration[26][27][28]. This problem was recognized previously for passive samplers called semipermeable membrane devices (SPMDs, e.g. polyethylene bags filled with triolein[29]) that were deployed in surface waters. As a result, representative chemicals called performance reference compound (PRCs) were dosed inside the samplers before their deployment in the environment, and the PRCs' diffusive losses out of the SPMD could be used to quantify the fractional approach toward sampler-environmental surroundings equilibration[30][29]. A similar approach can be used for polymers inserted in sediment beds[26][31]. Commonly, isotopically labeled forms of the compounds of interest such as deuterated or 13C-labelled PAHs or PCBs are homogeneously impregnated into the polymers before their deployments. Upon insertion of the polymer into the sediment bed (or overlying waters or even air), the initially evenly distributed PRCs begin to diffuse out of the sampling polymer and into the surroundings (Figure 2).
Assuming the contaminants of interest undergo the same mass transfer restrictions limiting their rates of uptake into the polymer (e.g., diffusion through the sedimentary porous medium) that are also limiting transfers of the PRCs out of the polymer[26][31], then fractional losses of the PRCs during a particular deployment can be used to adjust the accumulated contaminant loads to what they would have been at equilibrium with their surroundings with Equation 4.
Equation 4. | C(∞)polymer = C(t)polymer / fPRC lost | |
where: | ||
fPRC lost | is the fraction of the PRC lost to outward diffusion, | |
C(∞)polymer | is the concentration of the contaminant in the polymer at equilibrium, and | |
C(t)polymer | is the concentration of the contaminant in the polymer after deployment time, t. |
Since investigators are commonly interested in many chemicals at the same time, it is impractical to have a PRC for each contaminant of interest. Instead, a representative set of PRCs is used to characterize the rates of polymer-environment exchange as a function of the PRCs' properties (e.g., diffusivities, partition coefficients), the sediments characteristics (e.g., porosity), and the nature of the polymer used (e.g., film thickness, affinity for the chemicals)[26][27]. The resulting mass transfer model fit can then be used to estimate the fractional approaches to equilibrium for many other contaminants, whose diffusive and partitioning properties are also known. And these fractions can be used to adjust the target chemical concentrations that have accumulated from the sediment into the same polymeric sampler to find the equilibrated results[31]. Finally, these equilibrated concentrations can be used in Eq. 2 to estimate truly dissolved contaminant concentrations in the sediment's porewater.
Field Applications
Polymeric materials can be deployed in sediment in various ways[32]. PDMS coatings can be incorporated into slotted silica rods called SPMEs (solid phase micro extraction devices), while thin sheets of polymers like LDPE or POM can be incorporated into sheet metal frames. In both cases, such hardware is used to insert the polymers into sediment beds (Figure 3).
Deployment of the assembled passive samplers can be accomplished via poles from a boat[31], by divers[23], or by attaching the samplers to a sampling platform lowered off a vessel[33]. Typically, the method used depends on the water depth. Small buoys on short lines, sometimes with associated water-sampling polymeric materials in mesh bags (see right panel of Figure 3), are attached to the samplers to facilitate the sampler recoveries. After recovery, the samplers are wiped to remove any adhering sediment, biofilm, or precipitates and returned to the laboratory for PRC and target contaminant analyses. The resulting measurements of the accumulated target chemical concentrations can be adjusted using the observed PRC losses and publicly available software programs[34][35].
Subsequently, since the passive sampling reveals the concentrations of contaminants in a sediment bed's porewater and the overlying bottom water[18], the data can be used to estimate bed-to-water column diffusive fluxes of contaminants[36][33] and bioirrigation-affected fluxes[37]. The data are also useful for assessing the tendency of the contaminants to accumulate in benthic organisms[38][39][40], and by extension into food webs that include such benthic species[41]. Furthermore, recent efforts have found that passive sampling observations can be used to infer in situ transformations of substances like nitro aromatic compounds[42] and DDT[43].
References
- ^ 1.0 1.1 1.2 1.3 Kotamarthi, R., Mearns, L., Hayhoe, K., Castro, C.L., and Wuebble, D., 2016. Use of Climate Information for Decision-Making and Impacts Research: State of Our Understanding. Department of Defense, Strategic Environmental Research and Development Program (SERDP), 55pp. Free download from: SERDP-ESTCP
- ^ Kilgore, R., Thomas, W.O. Jr., Douglass, S., Webb, B., Hayhoe, K., Stoner, A., Jacobs, J.M., Thompson, D.B., Herrmann, G.R., Douglas, E., and Anderson, C., 2019. Applying Climate Change Information to Hydrologic and Coastal Design of Transportation Infrastructure, Design Practices. The National Cooperative Highway Research Program, Transportation Research Board, Project 15-61, 154 pages. Free download from: The Transportation Research Board
- ^ Maraun, D., and Wildmann, M., 2018. Statistical Downscaling and Bias Correction for Climate Research. Cambridge University Press, Cambridge, UK. 347 pages. DOI: 10.1017/9781107588783 ISBN: 978-1-107-06605-2
- ^ 4.0 4.1 Kotamarthi, R., Hayhoe, K., Wuebbles, D., Mearns, L.O., Jacobs, J. and Jurado, J., 2021. Downscaling Techniques for High-Resolution Climate Projections: From Global Change to Local Impacts. Cambridge University Press, Cambridge, UK. 202 pages. DOI: 10.1017/9781108601269 ISBN: 978-1-108-47375-0
- ^ New York State Department of Environmental Conservation, 2020. New York State Flood Risk Management Guidance for Implementation of the Community Risk and Resiliency Act. Free download from: New York State Report.pdf
- ^ US Department of Defense, 2019. Report on Effects of a Changing Climate to the Department of Defense. Free download from: DoD Report.pdf
- ^ Brownawell, B.J., and Farrington, J.W., 1986. Biogeochemistry of PCBs in interstitial waters of a coastal marine sediment. Geochimica et Cosmochimica Acta, 50(1), pp. 157-169. DOI: 10.1016/0016-7037(86)90061-X Free download available from: US EPA.
- ^ Chin, Y.P., and Gschwend, P.M., 1992. Partitioning of Polycyclic Aromatic Hydrocarbons to Marine Porewater Organic Colloids. Environmental Science and Technology, 26(8), pp. 1621-1626. DOI: 10.1021/es00032a020
- ^ Achman, D.R., Brownawell, B.J., and Zhang, L., 1996. Exchange of Polychlorinated Biphenyls Between Sediment and Water in the Hudson River Estuary. Estuaries, 19(4), pp. 950-965. DOI: 10.2307/1352310 Free download available from: Academia.edu
- ^ 10.0 10.1 Gustafsson, Ö., Haghseta, F., Chan, C., MacFarlane, J., and Gschwend, P.M., 1996. Quantification of the Dilute Sedimentary Soot Phase: Implications for PAH Speciation and Bioavailability. Environmental Science and Technology, 31(1), pp. 203-209. DOI: 10.1021/es960317s
- ^ Luthy, R.G., Aiken, G.R., Brusseau, M.L., Cunningham, S.D., Gschwend, P.M., Pignatello, J.J., Reinhard, M., Traina, S.J., Weber, W.J., and Westall, J.C., 1997. Sequestration of Hydrophobic Organic Contaminants by Geosorbents. Environmental Science and Technology, 31(12), pp. 3341-3347. DOI: 10.1021/es970512m
- ^ Lohmann, R., MacFarlane, J.K., and Gschwend, P.M., 2005. Importance of Black Carbon to Sorption of Native PAHs, PCBs, and PCDDs in Boston and New York Harbor Sediments. Environmental Science and Technology, 39(1), pp.141-148. DOI: 10.1021/es049424+
- ^ Cornelissen, G., Gustafsson, Ö., Bucheli, T.D., Jonker, M.T., Koelmans, A.A., and van Noort, P.C., 2005. Extensive Sorption of Organic Compounds to Black Carbon, Coal, and Kerogen in Sediments and Soils: Mechanisms and Consequences for Distribution, Bioaccumulation, and Biodegradation. Environmental Science and Technology, 39(18), pp. 6881-6895. DOI: 10.1021/es050191b
- ^ Koelmans, A.A., Kaag, K., Sneekes, A., and Peeters, E.T.H.M., 2009. Triple Domain in Situ Sorption Modeling of Organochlorine Pesticides, Polychlorobiphenyls, Polyaromatic Hydrocarbons, Polychlorinated Dibenzo-p-Dioxins, and Polychlorinated Dibenzofurans in Aquatic Sediments. Environmental Science and Technology, 43(23), pp. 8847-8853. DOI: 10.1021/es9021188
- ^ Schwarzenbach, R.P., Gschwend, P.M., and Imboden, D.M., 2017. Environmental Organic Chemistry, 3rd edition. Ch. 16: Equilibrium Partitioning from Water and Air to Biota, pp. 469-521. John Wiley and Sons. ISBN: 978-1-118-76723-8
- ^ Hawthorne, S.B., Grabanski, C.B., Miller, D.J., and Kreitinger, J.P., 2005. Solid-Phase Microextraction Measurement of Parent and Alkyl Polycyclic Aromatic Hydrocarbons in Milliliter Sediment Pore Water Samples and Determination of KDOC Values. Environmental Science and Technology, 39(8), pp. 2795-2803. DOI: 10.1021/es0405171
- ^ Mayer, P., Vaes, W.H., Wijnker, F., Legierse, K.C., Kraaij, R., Tolls, J., and Hermens, J.L., 2000. Sensing Dissolved Sediment Porewater Concentrations of Persistent and Bioaccumulative Pollutants Using Disposable Solid-Phase Microextraction Fibers. Environmental Science and Technology, 34(24), pp. 5177-5183. DOI: 10.1021/es001179g
- ^ 18.0 18.1 Booij, K., Hoedemaker, J.R., and Bakker, J.F., 2003. Dissolved PCBs, PAHs, and HCB in Pore Waters and Overlying Waters of Contaminated Harbor Sediments. Environmental Science and Technology, 37(18), pp. 4213-4220. DOI: 10.1021/es034147c
- ^ Cornelissen, G., Pettersen, A., Broman, D., Mayer, P., and Breedveld, G.D., 2008. Field testing of equilibrium passive samplers to determine freely dissolved native polycyclic aromatic hydrocarbon concentrations. Environmental Toxicology and Chemistry, 27(3), pp. 499-508. DOI: 10.1897/07-253.1
- ^ Tomaszewski, J.E., and Luthy, R.G., 2008. Field Deployment of Polyethylene Devices to Measure PCB Concentrations in Pore Water of Contaminated Sediment. Environmental Science and Technology, 42(16), pp. 6086-6091. DOI: 10.1021/es800582a
- ^ Fernandez, L.A., MacFarlane, J.K., Tcaciuc, A.P., and Gschwend, P.M., 2009. Measurement of Freely Dissolved PAH Concentrations in Sediment Beds Using Passive Sampling with Low-Density Polyethylene Strips. Environmental Science and Technology, 43(5), pp. 1430-1436. DOI: 10.1021/es802288w
- ^ Arp, H.P.H., Hale, S.E., Elmquist Kruså, M., Cornelissen, G., Grabanski, C.B., Miller, D.J., and Hawthorne, S.B., 2015. Review of polyoxymethylene passive sampling methods for quantifying freely dissolved porewater concentrations of hydrophobic organic contaminants. Environmental Toxicology and Chemistry, 34(4), pp. 710-720. DOI: 10.1002/etc.2864 Free access article. Report.pdf
- ^ 23.0 23.1 Cite error: Invalid
<ref>
tag; no text was provided for refs namedApell2016
- ^ Lohmann, R., 2012. Critical Review of Low-Density Polyethylene’s Partitioning and Diffusion Coefficients for Trace Organic Contaminants and Implications for Its Use as a Passive Sampler. Environmental Science and Technology, 46(2), pp. 606-618. DOI: 10.1021/es202702y
- ^ Ghosh, U., Kane Driscoll, S., Burgess, R.M., Jonker, M.T., Reible, D., Gobas, F., Choi, Y., Apitz, S.E., Maruya, K.A., Gala, W.R., Mortimer, M., and Beegan, C., 2014. Passive Sampling Methods for Contaminated Sediments: Practical Guidance for Selection, Calibration, and Implementation. Integrated Environmental Assessment and Management, 10(2), pp. 210-223. DOI: 10.1002/ieam.1507 Free access article. Report.pdf
- ^ 26.0 26.1 26.2 26.3 Fernandez, L. A., Harvey, C.F., and Gschwend, P.M., 2009. Using Performance Reference Compounds in Polyethylene Passive Samplers to Deduce Sediment Porewater Concentrations for Numerous Target Chemicals. Environmental Science and Technology, 43(23), pp. 8888-8894. DOI: 10.1021/es901877a
- ^ 27.0 27.1 Lampert, D.J., Thomas, C., and Reible, D.D., 2015. Internal and external transport significance for predicting contaminant uptake rates in passive samplers. Chemosphere, 119, pp. 910-916. DOI: 10.1016/j.chemosphere.2014.08.063 Free download available from: Academia.edu
- ^ Apell, J.N., Tcaciuc, A.P., and Gschwend, P.M., 2016. Understanding the rates of nonpolar organic chemical accumulation into passive samplers deployed in the environment: Guidance for passive sampler deployments. Integrated Environmental Assessment and Management, 12(3), pp. 486-492. DOI: 10.1002/ieam.1697
- ^ 29.0 29.1 Huckins, J.N., Petty, J.D., Lebo, J.A., Almeida, F.V., Booij, K., Alvarez, D.A., Cranor, W.L., Clark, R.C., and Mogensen, B.B., 2002. Development of the Permeability/Performance Reference Compound Approach for In Situ Calibration of Semipermeable Membrane Devices. Environmental Science and Technology, 36(1), pp. 85-91. DOI: 10.1021/es010991w
- ^ Booij, K., Smedes, F., and van Weerlee, E.M., 2002. Spiking of performance reference compounds in low density polyethylene and silicone passive water samplers. Chemosphere 46(8), pp.1157-1161. DOI: 10.1016/S0045-6535(01)00200-4
- ^ 31.0 31.1 31.2 31.3 Cite error: Invalid
<ref>
tag; no text was provided for refs namedApell2014
- ^ Cite error: Invalid
<ref>
tag; no text was provided for refs namedBurgess2017
- ^ 33.0 33.1 Fernandez, L.A., Lao, W., Maruya, K.A., White, C., Burgess, R.M., 2012. Passive Sampling to Measure Baseline Dissolved Persistent Organic Pollutant Concentrations in the Water Column of the Palos Verdes Shelf Superfund Site. Environmental Science and Technology, 46(21), pp. 11937-11947. DOI: 10.1021/es302139y
- ^ Gschwend, P.M., Tcaciuc, A.P., and Apell, J.N., 2014. Guidance Document: Passive PE Sampling in Support of In Situ Remediation of Contaminated Sediments – Passive Sampler PRC Calculation Software User’s Guide, US Department of Defense, Environmental Security Technology Certification Program Project ER-200915. Available from: ESTCP.
- ^ Thompson, J.M., Hsieh, C.H. and Luthy, R.G., 2015. Modeling Uptake of Hydrophobic Organic Contaminants into Polyethylene Passive Samplers. Environmental Science and Technology, 49(4), pp. 2270-2277. DOI: 10.1021/es504442s
- ^ Koelmans, A.A., Poot, A., De Lange, H.J., Velzeboer, I., Harmsen, J., and van Noort, P.C.M., 2010. Estimation of In Situ Sediment-to-Water Fluxes of Polycyclic Aromatic Hydrocarbons, Polychlorobiphenyls and Polybrominated Diphenylethers. Environmental Science and Technology, 44(8), pp. 3014-3020. DOI: 10.1021/es903938z
- ^ Apell, J.N., Shull, D.H., Hoyt, A.M., and Gschwend, P.M., 2018. Investigating the Effect of Bioirrigation on In Situ Porewater Concentrations and Fluxes of Polychlorinated Biphenyls Using Passive Samplers. Environmental Science and Technology, 52(8), pp. 4565-4573. DOI: 10.1021/acs.est.7b05809
- ^ Vinturella, A.E., Burgess, R.M., Coull, B.A., Thompson, K.M., and Shine, J.P., 2004. Use of Passive Samplers to Mimic Uptake of Polycyclic Aromatic Hydrocarbons by Benthic Polychaetes. Environmental Science and Technology, 38(4), pp. 1154-1160. DOI: 10.1021/es034706f
- ^ Yates, K., Pollard, P., Davies, I.M., Webster, L., and Moffat, C.F., 2011. Application of silicone rubber passive samplers to investigate the bioaccumulation of PAHs by Nereis virens from marine sediments. Environmental Pollution, 159(12), pp. 3351-3356. DOI: 10.1016/j.envpol.2011.08.038
- ^ Fernandez, L.A. and Gschwend, P.M., 2015. Predicting bioaccumulation of polycyclic aromatic hydrocarbons in soft-shelled clams (Mya arenaria) using field deployments of polyethylene passive samplers. Environmental Toxicology and Chemistry, 34(5), pp. 993-1000. DOI: 10.1002/etc.2892
- ^ von Stackelberg, K., Williams, M.A., Clough, J., and Johnson, M.S., 2017. Spatially explicit bioaccumulation modeling in aquatic environments: Results from 2 demonstration sites. Integrated Environmental Assessment and Management, 13(6), pp. 1023-1037. DOI: 10.1002/ieam.1927
- ^ Belles, A., Alary, C., Criquet, J., and Billon, G., 2016. A new application of passive samplers as indicators of in-situ biodegradation processes. Chemosphere, 164, pp. 347-354. DOI: 10.1016/j.chemosphere.2016.08.111
- ^ Tcaciuc, A.P., Borrelli, R., Zaninetta, L.M., and Gschwend, P.M., 2018. Passive sampling of DDT, DDE and DDD in sediments: accounting for degradation processes with reaction–diffusion modeling. Environmental Science: Processes and Impacts, 20(1), pp. 220-231. DOI: 10.1039/C7EM00501F Open access article available from: Royal Society of Chemistry.