Zerovalent Iron Permeable Reactive Barriers
Permeable reactive barriers (PRBs) are in situ treatment zones created below ground to clean up contaminated groundwater. PRBs take advantage of natural groundwater migration to transport contaminants to a defined treatment zone. Contaminants are removed from groundwater in the PRB as treated groundwater passes through the permeable zone. Eventually a “clean front” is created on the down-gradient side of the PRB. Zerovalent Iron (ZVI) was the first reactive material used in PRBs for groundwater remediation, and it continues to be the primary material used in the construction of these treatment systems. ZVI PRBs can treat groundwater contaminated with chlorinated solvents and their breakdown products such as tetrachloroethene (PCE), trichloroethene (TCE), cis-1,2-dichloroethene (cis-DCE), vinyl chloride (VC), 1,1,1-trichloroethane (1,1,1-TCA), 1,1-dichloroethane (1,1-DCA), 1,2-dichloroethane (1,2-DCA), chloroform, and carbon tetrachloride; also explosives such as TNT and RDX; as well as cations of Pb, Cd, Ni, Zn, and Hg; and anions of Cr, As, Sb, Se, U, and Tc.
Related Article(s):
- Zerovalent Iron (ZVI) (Chemical Reduction - ISCR)
- Chemical Reduction (In Situ - ISCR)
- Metal and Metalloids - Remediation
- Chlorinated Solvents
Contributor(s): Dr. Richard Wilkin
Key Resource(s):
- Permeable Reactive Barriers: Lessons Learned/New Directions. Interstate Technology Regulatory Council (2005)[1].
- Permeable Reactive Barrier: Technology Update. Interstate Technology Regulatory Council (2011)[2]
Introduction
A PRB is commonly constructed using granular iron, limestone, organic-carbon, zeolites, apatite, or mixtures of these materials with sand or gravel[1][2]. PRBs are installed hydraulically down-gradient from contaminant source zones to prevent off-site plume migration or to protect sensitive receptors. Design considerations for PRBs include:
- understanding contaminant flux to determine the residence time requirement for effective treatment;
- understanding geochemical conditions to identify compatibility issues between the selected reactive medium and site-specific groundwater; and
- understanding groundwater flow to ensure the contaminant plume is intercepted for treatment (See Figure 1) so contaminant flow beneath, around, or above the treatment system does not occur. PRBs are generally keyed into impermeable hydrostratigraphic units, such as clay layers or bedrock, to prevent underflow of contaminants beneath the treatment zone.
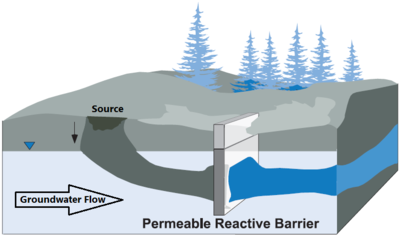
Zerovalent Iron
The first field-scale test of a PRB constructed with ZVI was conducted in 1991 at the Canadian Forces Base, Borden, Ontario by researchers from the University of Waterloo[4][5]. The first full-scale ZVI-based PRB was constructed in Sunnyvale, California in 1994 to treat groundwater contaminated with TCE and cis-DCE[6]. Long-term performance studies have documented sustained removal of trichloroethene and hexavalent chromium by ZVI PRBs and their continued hydraulic performance for up to 15 years[6][7][8].
ZVI degrades chlorinated ethenes, ethanes, and methanes via reductive abiotic reactions that largely circumvent the production of toxic chlorinated daughter compounds, such as vinyl chloride, which can be produced during biological reduction[9][10]. For example, Arnold and Roberts[10] reported that >85% of PCE and >90% of TCE treatment by zerovalent iron is accounted for by β-elimination reactions that avoid production of cis-DCE and VC.
Contaminant Class | Contaminant | Removal Mechanism |
---|---|---|
Chlorinated Ethenes | Tetrachloroethene, PCE Trichloroethene, TCE cis-1,2-Dichloroethene, cis-DCE trans-1,2-Dichloroethene, trans-DCE 1,1- Dichloroethene, 1,1-DCE Vinyl Chloride, VC |
α-elimination β-elimination Hydrogenolysis |
Chlorinated Ethanes | Hexachloroethane, HCA 1,1,1,2-Tetrachloroethane, 1,1,1,2-TeCA 1,1,2,2-Tetrachloroethane, 1,1,2,2-TeCA 1,1,1-Trichloroethane, 1,1,1-TCA 1,1-Dichloroethane, 1,1-DCA |
α-elimination β-elimination Hydrogenolysis |
Chlorinated Methanes | Tetrachloromethane, CT Trichloromethane, TCM |
α-elimination β-elimination Hydrogenolysis |
Explosives | Trinitrotoluene (TNT) Hexahydro-1,3,5-trinitro-1,3,5-triazine (RDX) |
Adsorption Reduction to 2,4,6-triaminotoluene (TAT) |
Anionic Metals | Chromium Arsenic Antimony Selenium Uranium Technetium |
Reductive precipitation Co-precipitation Adsorption |
Metals | Lead Cadmium Nickel Zinc Mercury |
Precipitation Co-precipitation Adsorption |
Zerovalent Iron has also been used in pilot or full-scale tests to treat groundwater contaminated with metals (e.g., chromium), metalloids (e.g., arsenic), and radionuclides (e.g., uranium)[11][12][13][14]. Contaminant removal processes are complex and involve a combination of sorption to the surface of the ZVI or secondary minerals formed in the PRB, reductive precipitation, and co-precipitation. Other possible uses of ZVI for groundwater include treatment of explosives such as nitro aromatic compounds[15][16]. Treatment applications of ZVI for organic and inorganic contaminants encountered in groundwater at hazardous waste sites are summarized in Table 1.
PRB Design and Site Conditions
Like other in-situ technologies used for groundwater cleanup, PRB designs rely on accurate site characterization data for geology, hydrology, and groundwater geochemistry. Site hydrologic aspects critical for PRB design and performance include seasonal consistency in groundwater flow direction to maintain migration of contaminants to the PRB at an appropriate flow velocity. High flow velocities (≳2 m/d) can result in short residence time in the treatment zone and early breakthrough of contaminants; low flow velocities (≲0.1 m/d) result in little change in contaminant concentrations over time, so wells in down-gradient regions respond slowly. Geochemical considerations for the design of ZVI PRBs include redox conditions and the concentrations of major ions dissolved in local groundwater. Geochemical conditions that are favorable for the sustained performance of ZVI PRBs include:
- Dissolved O2 concentrations ≲2 mg/L: Excess dissolved oxygen leads to rapid iron corrosion and formation of low density iron oxide precipitates that may lead to hydraulic failure.
- Nitrate-N concentrations ≲10 mg/L: Like dissolved oxygen, nitrate enhanced iron corrosion leads to rapid iron corrosion and formation of low density iron oxide precipitates that may lead to hydraulic failure[17].
- Bicarbonate alkalinity ≲250 mg CaCO3/L: Carbonate minerals (aragonite, siderite, chukanovite, carbonate-green rust) precipitate in the moderately alkaline pH environment (pH~10) produced by ZVI PRBs and most of the porosity and reactivity reduction in PRBs can be accounted for by these minerals (see Figure 2)[18][19][20][21].
- Sulfate concentrations ≳100 mg/L: High dissolved hydrogen generated by iron corrosion creates a favorable environment for sulfate-reducing bacteria and methane-producing archaea. Metal sulfide precipitates like mackinawite (FeS) provide a source of secondary reactivity for chlorinated solvents and metals[22][23]. Thus, levels of sulfate in influent groundwater promote natural sulfidation of ZVI PRBs and potentially enhanced treatment capacity[24].
- Total dissolved solids below ≲1500 mg/L: The amount of secondary mineralization and clogging potential increases with increasing solute levels.
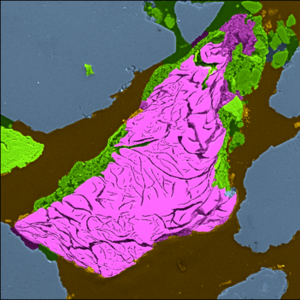
Performance Monitoring
Performance monitoring of PRBs typically includes: 1) sampling groundwater monitoring wells located up-gradient of the PRB to establish influent contaminant concentrations and groundwater geochemistry; 2) sampling groundwater monitoring wells located within the PRB to establish in-wall behavior; and, 3) sampling groundwater monitoring wells located down-gradient of the PRB to establish contaminant concentrations and groundwater geochemistry in the effluent. Key monitoring parameters include: contaminant(s) and daughter product(s), pH, oxidation-reduction potential, specific conductance, alkalinity, calcium, iron, and sulfate. These monitoring parameters provide an indication of whether or not the expected geochemical environment is present within the treatment zone, and also that contaminant degradation is occurring.
For chlorinated solvents, analysis of the parent compounds and daughter products (e.g., ethene and ethane) aids in the performance analysis. Compound Specific Isotope Analysis (CSIA) can be helpful to understand contaminant degradation processes in complicated systems[26][27]. Hydrologic monitoring typically consists of gradient analysis to determine flow directions and whether water level changes are abrupt in the vicinity of the PRB, indicating the potential for impeded groundwater flow. Core collection with solid-phase analysis and/or geophysical surveys (e.g., conductivity probing) are not common or routine components of performance monitoring, but can be considered in situations where anomalous behavior is encountered such as a failure to meet performance criteria.
Problems noted with the application of this, and other, in situ technologies include:
- contaminants are present down-gradient of the PRB prior to installation;
- influent contaminant concentrations may increase with time such that necessary residence time requirements are not met;
- incomplete capture of the plume, especially along peripheries of the plume;
- unaccounted for high contaminant flux zones and early breakthrough; and,
- incomplete coverage of the reactive medium in the subsurface.
Many of these issues can be addressed with rigorous site characterization, which may incur extra cost and time prior to remediation, but can potentially avoid implementation of secondary measures.
Summary & Outlook
ZVI is perhaps the most widely studied and best understood reactive material used for groundwater remediation. It will continue to serve as a benchmark for other novel treatment media and for studying treatment options for emerging contaminants. The environmental industry is becoming accustomed to screening sites for potential ZVI applications and is actively taking advantage of improving ZVI performance, i.e., through natural sulfidation and treating up-gradient sources of contamination to extend the lifetime of the PRB. Advantages of using ZVI in PRBs for remediating contaminated groundwater include: wide range of treatable contaminants (organics and inorganics); expectation of complete subsurface coverage; and, flexible adaptation to variable contaminant flux and plume geometry. Disadvantages include: construction challenges and uncertainty in predicting long-term treatment and hydraulic performance. These uncertainties can often be addressed with rigorous site characterization.
References
- ^ 1.0 1.1 Interstate Technology & Regulatory Council (ITRC), 2005. Permeable Reactive Barriers: Lessons Learned/New Directions. PRB-4. Washington, D.C.: Interstate Technology & Regulatory Council, Permeable Reactive Barriers Team. Report.pdf
- ^ 2.0 2.1 Interstate Technology & Regulatory Council (ITRC), 2011. Permeable Reactive Barrier: Technology Update. PRB-5. Washington, D.C.: Interstate Technology & Regulatory Council, PRB: Technology Update Team. Report.pdf
- ^ Wilkin, R T., Puls, R W. P and Sewell, G W. Environmental Research Brief: Long-term Performance of Permeable Reactive Barriers Using Zero-valent Iron: An Evaluation at Two Sites. US EPAU.S. Environmental Protection Agency, Washington, DC, EPA/600/S-02/001, 2002. Report.pdf
- ^ Gillham, R.W. and O'Hannesin, S.F., 1994. Enhanced degradation of halogenated aliphatics by zero‐valent iron. Groundwater, 32(6), pp.958-967. doi: 10.1111/j.1745-6584.1994.tb00935.x
- ^ O'Hannesin, S.F. and Gillham, R.W., 1998. Long‐term performance of an in situ “iron wall” for remediation of VOCs. Groundwater, 36(1), pp.164-170. doi: 10.1111/j.1745-6584.1998.tb01077.x
- ^ 6.0 6.1 Warner, S.D., Longino, B.L., Zhang, M.I.A.O., Bennett, P., Szerdy, F.S. and Hamilton, L.A., 2005. The first commercial permeable reactive barrier composed of granular iron: hydraulic and chemical performance at 10 years of operation. IAHS Publication, 298, p.32 - 42. ISBN 1-901502-23-6]
- ^ Phillips, D.H., Nooten, T.V., Bastiaens, L., Russell, M.I., Dickson, K., Plant, S., Ahad, J.M.E., Newton, T., Elliot, T. and Kalin, R.M., 2010. Ten year performance evaluation of a field-scale zero-valent iron permeable reactive barrier installed to remediate trichloroethene contaminated groundwater. Environmental Science & Technology, 44(10), pp.3861-3869. doi: 10.1021/es902737t
- ^ Wilkin, R.T., Acree, S.D., Ross, R.R., Puls, R.W., Lee, T.R. and Woods, L.L., 2014. Fifteen-year assessment of a permeable reactive barrier for treatment of chromate and trichloroethylene in groundwater. Science of the Total Environment, 468, pp.186-194. doi: 10.1016/j.scitotenv.2013.08.056
- ^ Matheson, L.J. and Tratnyek, P.G., 1994. Reductive dehalogenation of chlorinated methanes by iron metal. Environmental Science & Technology, 28(12), pp.2045-2053. doi:10.1021/es00061a012
- ^ 10.0 10.1 Arnold, W.A. and Roberts, A.L., 2000. Pathways and kinetics of chlorinated ethylene and chlorinated acetylene reaction with Fe (0) particles. Environmental Science & Technology, 34(9), pp.1794-1805. doi: 10.1021/es990884q
- ^ Blowes, D.W., Ptacek, C.J., Benner, S.G., McRae, C.W., Bennett, T.A. and Puls, R.W., 2000. Treatment of inorganic contaminants using permeable reactive barriers. Journal of Contaminant Hydrology, 45(1), pp.123-137
- ^ Puls, R.W., Paul, C.J. and Powell, R.M., 1999. The application of in situ permeable reactive (zero-valent iron) barrier technology for the remediation of chromate-contaminated groundwater: a field test. Applied Geochemistry, 14(8), pp.989-1000. doi: 10.1016/s0883-2927(99)00010-4
- ^ Wilkin, R.T., Acree, S.D., Ross, R.R., Beak, D.G. and Lee, T.R., 2009. Performance of a zerovalent iron reactive barrier for the treatment of arsenic in groundwater: Part 1. Hydrogeochemical studies. Journal of Contaminant Hydrology, 106(1-2), pp.1-14. doi: 10.1016/j.jconhyd.2008.12.002
- ^ Morrison, S.J., Metzler, D.R. and Carpenter, C.E., 2001. Uranium precipitation in a permeable reactive barrier by progressive irreversible dissolution of zerovalent iron. Environmental Science & Technology, 35(2), pp.385-390. doi: 10.1021/es001204i
- ^ Agrawal, A. and Tratnyek, P.G., 1995. Reduction of nitro aromatic compounds by zero-valent iron metal. Environmental Science & Technology,30(1), pp.153-160. doi:10.1021/es950211h
- ^ Scherer, M.M., Johnson, K.M., Westall, J.C. and Tratnyek, P.G., 2001. Mass transport effects on the kinetics of nitrobenzene reduction by iron metal. Environmental Science & Technology, 35(13), pp.2804-2811. doi: 10.1021/es0016856
- ^ Liang, L., Moline, G.R., Kamolpornwijit, W. and West, O.R., 2005. Influence of hydrogeochemical processes on zero-valent iron reactive barrier performance: A field investigation. Journal of Contaminant Hydrology, 78(4), pp.291-312. doi: 10.1016/j.jconhyd.2005.05.006
- ^ Jeen, S.W., Gillham, R.W. and Blowes, D.W., 2006. Effects of carbonate precipitates on long-term performance of granular iron for reductive dechlorination of TCE. Environmental Science & Technology, 40(20), pp.6432-6437. doi: 10.1021/es0608747
- ^ Li, L., Benson, C.H. and Lawson, E.M., 2006. Modeling porosity reductions caused by mineral fouling in continuous-wall permeable reactive barriers. Journal of Contaminant Hydrology, 83(1-2), pp.89-121. doi: 10.1016/j.jconhyd.2005.11.004
- ^ Parbs, A., Ebert, M. and Dahmke, A., 2007. Long-term effects of dissolved carbonate species on the degradation of trichloroethylene by zerovalent iron. Environmental Science & Technology, 41(1), pp.291-296. doi: 10.1021/es061397v
- ^ Henderson, A.D. and Demond, A.H., 2007. Long-term performance of zero-valent iron permeable reactive barriers: a critical review. Environmental Engineering Science, 24(4), pp.401-423. doi: 10.1089/ees.2006.0071
- ^ Butler, E.C. and Hayes, K.F., 1999. Kinetics of the transformation of trichloroethylene and tetrachloroethylene by iron sulfide. Environmental Science & Technology, 33(12), pp.2021-2027. doi: 10.1021/es9809455
- ^ Beak, D.G. and Wilkin, R.T., 2009. Performance of a zerovalent iron reactive barrier for the treatment of arsenic in groundwater: Part 2. Geochemical modeling and solid phase studies. Journal of Contaminant Hydrology, 106(1-2), pp.15-28. doi: 10.1016/j.jconhyd.2008.12.003
- ^ Fan, D., Lan, Y., Tratnyek, P.G., Johnson, R.L., Filip, J., O’Carroll, D.M., Nunez Garcia, A. and Agrawal, A., 2017. Sulfidation of iron-based materials: A review of processes and implications for water treatment and remediation. Environmental Science & Technology, 51(22), pp.13070-13085. doi: 10.1021/acs.est.7b04177
- ^ ESTCP, 2018. Analysis of Long-Term Performance of Zero-valent Iron Applications. Project Report #ER-201589-PR. Report.pdf
- ^ VanStone, N., Przepiora, A., Vogan, J., Lacrampe-Couloume, G., Powers, B., Perez, E., Mabury, S. and Lollar, B.S., 2005. Monitoring trichloroethene remediation at an iron permeable reactive barrier using stable carbon isotopic analysis. Journal of Contaminant Hydrology, 78(4), pp.313-325. doi: 10.1016/j.jconhyd.2005.05.013
- ^ Lojkasek‐Lima, P., Aravena, R., Shouakar‐Stash, O., Frape, S.K., Marchesi, M., Fiorenza, S. and Vogan, J., 2012. Evaluating TCE abiotic and biotic degradation pathways in a permeable reactive barrier using compound specific isotope analysis. Groundwater Monitoring & Remediation, 32(4), pp.53-62. doi: 10.1111/j.1745-6592.2012.01403.x